Review Article | Open Access
Effects of xenobiotics on the blood-brain barrier and neural gene expression
Mark Trussel1, Jiff Brad2
1Feik School of Pharmacy, University of the Incarnate Word, 4301 Broadway, San Antonio, TX 78209, USA.
2School of Health and Behavioral Sciences, University of the Sunshine Coast, 90 Sippy Downs Drive, Sippy Downs, Queensland 4556, Australia.
Correspondence: Mark Trussel (Feik School of Pharmacy, University of the Incarnate Word, 4301 Broadway, San Antonio, TX 78209, USA; E-mail: mark.trussel88@proton.me).
Asia-Pacific Journal of Pharmacotherapy & Toxicology 2024, 4: 47-54. https://doi.org/10.32948/ajpt.2024.09.20
Received: 17 Sep 2024 | Accepted: 28 Sep 2024 | Published online: 05 Oct 2024
Key words blood-brain barrier, xenobiotics, heavy metals, toxic compounds
Xenobiotics are chemical compounds that are foreign or unnatural to both human and animal systems. These substances encompass plant-derived components, pharmaceutical drugs, pesticides, cosmetic ingredients, artificial food flavors, fragrances, and more. Even naturally occurring compounds (endobiotics) can be classified as xenobiotics when present in elevated concentrations in environmental matrices [5]. Xenobiotics include pesticides, pharmaceutical compounds, personal care items, illicit drugs, industrial/commercial products, and nuclear waste, and can be found across various environmental media. These substances are utilized by humans and eventually make their way, directly or indirectly, into different environmental matrices, where they generate a range of metabolites and secondary products, some of which may be more toxic than the original compounds [6].
Xenobiotics can enter the body via inhalation, ingestion, and dermal absorption [7], making it difficult to control their exposure. When these substances infiltrate biological systems, they disrupt the homeostatic balance in the body, causing various effects, including gene alterations. These changes in genes can be either permanent or temporary. Xenobiotics influence gene expression by modulating epigenetic pathways, without any alterations to the DNA sequence [5, 8]. This in turn can lead to notable changes in brain function, with potential implications for neurological and neurodevelopmental disorders [9].
This review aims to examine the effects of various xenobiotics, such as heavy metals, on the BBB and brain function, focusing on the mechanisms by which these substances disrupt the BBB and the resulting functional and pathological changes in the brain.
Endothelial cells are the central anatomical component of the BBB, lining the cerebral blood vessels and interacting with various cell types within the CNS [18]. The endothelial cells of the BBB in the adult mammalian brain possess distinct characteristics that set them apart from ECs found in other parts of the body. They also differ from peripheral endothelial cells in function and morphology [19]. ECs are distinguished by their flattened shape, the presence of inter-endothelial tight junctions, a sparse number of caveolae on the luminal surface, and a higher concentration of mitochondria, especially when compared to endothelial cells from other vascular regions [12]. Endothelial cells are bound together by specialized TJs, which are significantly closer—50 to 100 times—than those found in peripheral capillaries. This proximity limits the passive movement of molecules into the brain and results in blood vessels having remarkably high transendothelial electrical resistance (TEER) [20]. These junctions are formed by claudin family proteins (Cldn) and occludin (Ocln), which are connected to the actin cytoskeleton through the ZO protein family (ZO-1, -2, -3) [21]. Any impairment in the regulation of these proteins compromises BBB integrity, allowing harmful substances into the brain and causing potential neurotoxicity or swelling [22]. Functionally, these cells possess a net negative surface charge, which hinders the binding of negatively charged molecules and leads to reduced expression of leukocyte adhesion molecules, limiting immune cell infiltration. Furthermore, they are equipped with specialized transporters that regulate the movement of specific substrates in and out of the cells. The number of transcellular vesicles traversing the vessel wall is minimized due to the high transendothelial electrical resistance [19].
Astrocytes, also referred to as astroglia, represent the largest population of glial cells and exhibit complex, polarized morphologies that vary across different brain regions [23]. Traditionally, they are classified into protoplasmic astrocytes, found in the well-vascularized gray matter, and fibrous astrocytes, located in the less vascularized white matter [24]. Astrocytes, known for their star-shaped structure, are highly prevalent and multifunctional cells that facilitate neuron migration during development and serve as buffers for potassium ions (K+) and neurotransmitters. These cells exhibit a stellate morphology with multiple extensions and are characterized by the expression of intermediate filament proteins such as vimentin (Vim) and glial fibrillary acidic protein (GFAP) [25]. Their end feet establish a connection with the basement membrane through the involvement of proteins such as aquaporin IV and the dystroglycan-dystrophin complex, which interact with the proteoglycan agrin [26-29]. In the CNS, they are vital for various functions, including waste clearance, blood flow regulation, vascular maintenance, ion balance, and neuroimmune response modulation [29, 30]. The junction between the astrocytic endfeet and the perivascular basal lamina is rich in organic anion transporters (OAPs), though their concentration decreases as the astrocytic membrane loses contact with the basal lamina [31]. This polarization of the astrocytic membrane is a notable structural feature of ACs in both mammals and birds, associated with the maturation of the BBB during development [12, 32].
Pericytes are cells that regulate blood-brain barrier development and function by controlling vascular permeability and inhibiting factors that increase immune cell infiltration into the CNS [33]. Pericytes play multiple roles in vascular function, such as controlling cerebral blood flow, supporting the integrity of the BBB, and guiding vascular development and angiogenesis. They are also capable of facilitating neuroinflammation and exhibit properties similar to stem cells [34]. Pericytes play a key role in the function of the neurovascular unit [35-37]. Their physical closeness to endothelial cells enables constant communication. One example of such interaction is the PDGF-B signaling pathway [38], where endothelial cells release PDGF-B to bind to PDGFRβ on pericytes, guiding their recruitment to the vasculature [39]. A decline in pericyte populations can disrupt tight junctions between ECs, thereby increasing the permeability of the BBB [40].
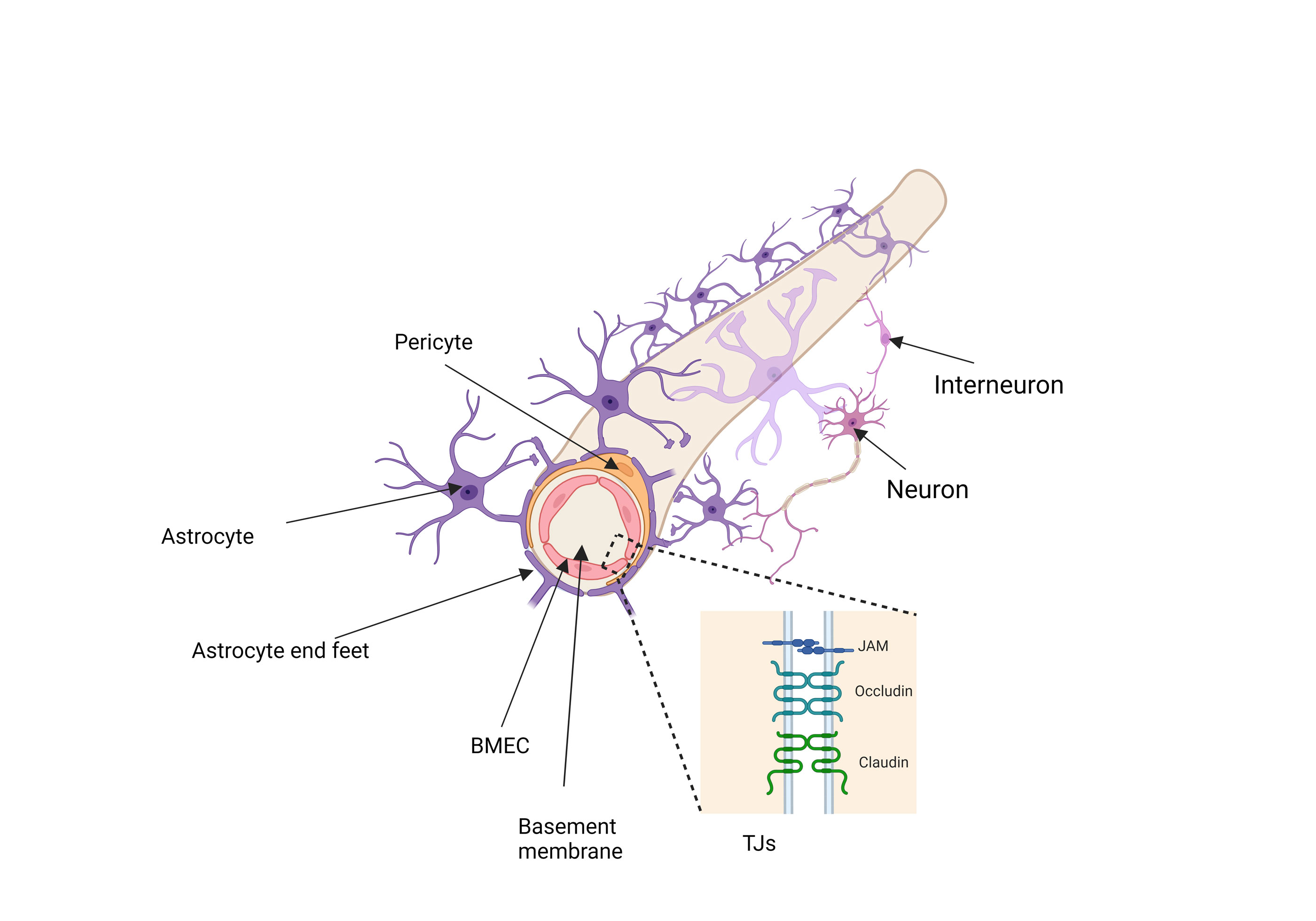
Another important component of BBB is adhering junctions, these junctions form distinct microdomains at cellular interfaces, characterized by their spatial, chemical, and mechanical properties [44]. Like tight junctions, adherens junctions are linked to the cytoskeleton and contain both transmembrane and cytoplasmic plaque proteins [45]. Adherens junctions play an importan role role in preserving the BBB, and any disruption to these junctions may impair the connections between endothelial cells [46].
Environmental factors including xenobiotics play a crucial role in epigenetic modulation, influencing cell differentiation both in early life and throughout the lifespan. These factors can actively regulate gene expression in a manner specific to different cells and tissues [63]. Genetic variations involve changes to the DNA sequence, whereas epigenetic regulation adjusts gene expression in response to environmental conditions, without altering the genome itself. Therefore, epigenetic mechanisms bridge the gap between environmental influences and genetic factors [64]. The epigenetic modification process involves chemical changes to chromatin and the regulation of miRNA expression [65]. Xenobiotics may exert broad systemic effects or target specific genomic regions, and they can influence the DNA methylome, histone modifications, or both. These compounds can either inhibit or enhance the activity of epigenetic modifiers. For instance, certain heavy metals impact DNMTs, histone deacetylases, and methyltransferases, ultimately regulating the epigenome. Additionally, xenobiotics may interfere with chemical moiety donors, such as arsenic disrupting SAM, the methyl group donor for DNA and histones, leading to epigenetic modifications [66, 67]. In some cases, these external substances can disrupt intermediary metabolism by altering levels of cofactors, co-enzymes, or substrate availability, or by affecting other enzymatic processes [68]. The epigenetic changes caused by xenobiotics may have either immediate or delayed effects, which could be transient or persist over the long term.
Tight junction proteins are particularly sensitive to environmental changes, and alterations in their microenvironment can lead to the dissociation of the occludin/ZO complex, which compromises the integrity of the BBB (Figure 2) [75]. A decrease in ZO-2 protein levels has been reported in cells exposed to Pb, further indicating altered BBB permeability in the model system studied [76]. Pb stimulation in C6 cells led to a decrease in ZO-1 (zonula occludens-1) and occludin protein levels in ECV304 cells [77]. Another investigation demonstrated that the TJ disruption is significantly influenced by the arsenic species and its chemical form [78]. The interaction of Cd with the vascular endothelium of the BBB led to the disruption of the TJ apparatus, which could subsequently cause secondary damage to the CNS [79]. Pb also upregulated the mRNA and protein expression of MMP-2/9 in C6 cells. By inhibiting MMP-2/9 with SB-3CT, we were able to partially reverse Pb-induced downregulation of tight junction proteins in ECV304 cells and reduce barrier impairment in the BBB model [77]. This suggests that the transport of these heavy metals may occur through various mechanisms, including the disruption of TJs.
Astrocytes are critical for maintaining homeostasis in the brain and executing a range of important tasks. They help regulate the blood-brain barrier, remove cellular debris, balance ions, control neurotransmitter levels, support neurogenesis and synaptogenesis, adjust synaptic connections, and release neurotrophins [80]. Astrocyte dysfunction can lead to the release of pro-inflammatory cytokines, destabilization of the blood-brain barrier, disruptions in glutamate and lipid metabolism, and eventually, the deterioration of synaptic integrity [81]. Astrocyte exposure to Cd, As and induced apoptosis by increasing the expression of peroxisome proliferator-activated receptor gamma (PPARγ) and promoting its interaction with the poly(ADP-ribose) polymerase (PARP) gene and PPARγ-response elements (PPREs) (Figure 2) [82].
A study reported that Pb is carried across an in vitro BBB model by a transporter with biochemical characteristics resembling those of the DMT1 isoform containing an iron-responsive element (IRE)[83]. Once it reaches BBB , Pb impairs endothelial cell function by reducing its viability (Figure 2). Exposure to Pb at concentrations of 3-100 µM exerted a cytotoxic effect on endothelial cells, inhibiting angiogenesis in a dose-dependent fashion. Pb disrupted normal EC physiological processes by interfering with the release of endogenous vascular protective mediators, TFPI-1 and TFPI-2. However, the negative impact of 3-30 µM Pb on the release of these mediators was successfully reversed by S-NACH in a concentration-dependent manner, restoring normal levels and mitigating Pb-induced angiogenesis disruption [84].
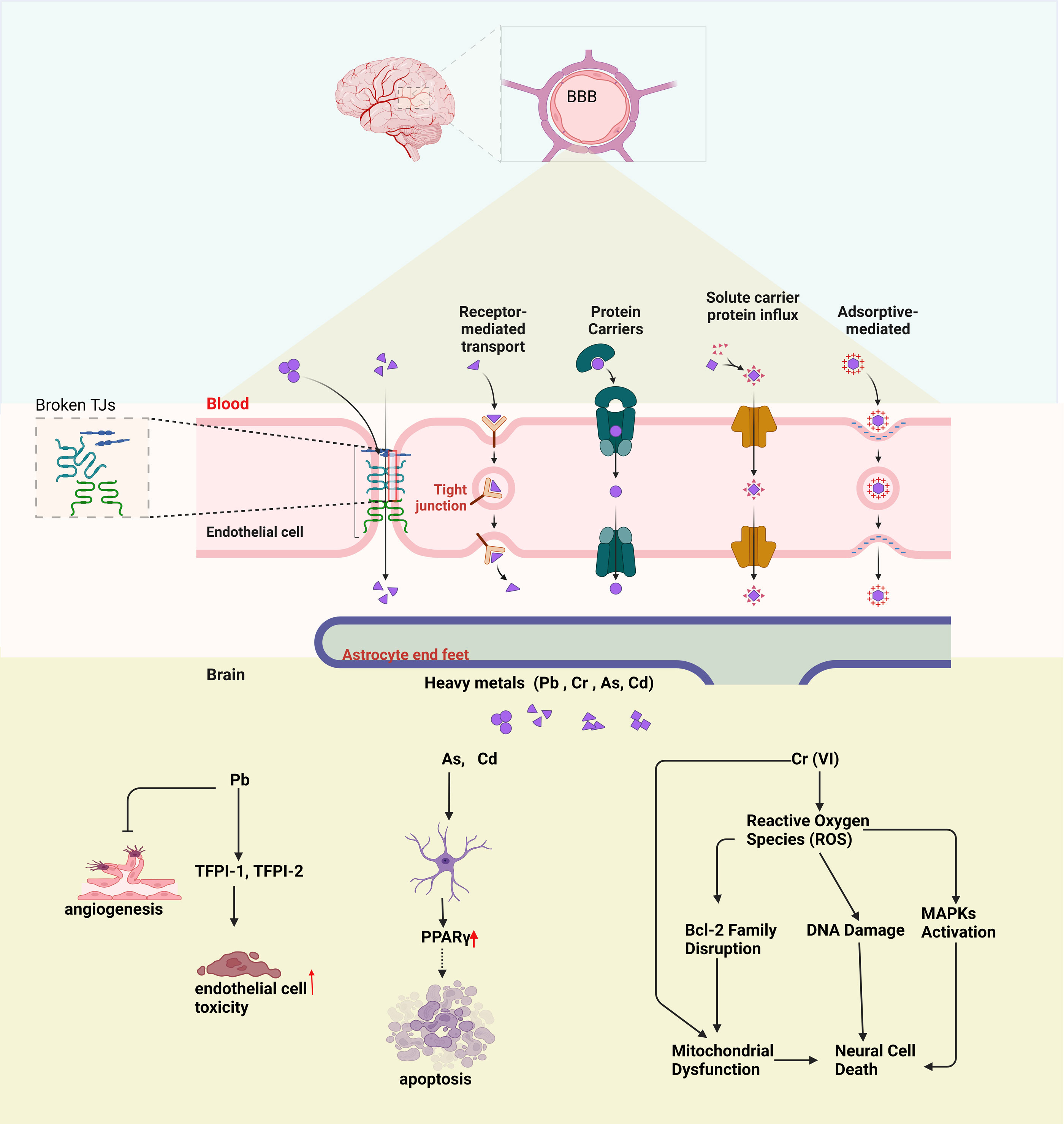
No applicable.
Ethics approval
No applicable.
Data availability
The data will be available upon request.
Funding
None.
Authors’ contribution
Mark Trussel and Jiff Brad contributed to draft, critical revision of the article. Mark Trussel approved the final version to be submited.
Competing interests
None.
- Benz F, Liebner S: Structure and function of the blood–brain barrier (BBB). Handb Exp Pharmacol 2022, 273: 3-31.
- Segarra M, Aburto MR, Acker-Palmer A: Blood–brain barrier dynamics to maintain brain homeostasis. Trends Neurosci 2021, 44(5): 393-405.
- Zha S, Liu H, Li H, Li H, Wong K-L, All AH: Functionalized nanomaterials capable of crossing the blood–brain barrier. ACS nano 2024, 18(3): 1820-1845.
- Pandit R, Chen L, Götz J: The blood-brain barrier: Physiology and strategies for drug delivery. Adv Drug Deliv Rev 2020, 165: 1-14.
- Balasubramanian S, Gunasekaran K, Sasidharan S, Mathan VJ, Perumal E: MicroRNAs and xenobiotic toxicity: an overview. Toxicol Rep 2020, 7: 583-595.
- Singh M, Mishra RC, Shah I, Wadhwa V, Mor V: Xenobiotics: Sources, Pathways, Degradation, and Risk Associated with Major Emphasis on Pharmaceutical Compounds. In: Xenobiotics in Urban Ecosystems: Sources, Distribution and Health Impacts. Epub ahead of print., edn.: Springer; 2023: 87-106.
- Ortiz P, Torres-Sánchez A, López-Moreno A, Cerk K, Ruiz-Moreno Á, Monteoliva-Sánchez M, Ampatzoglou A, Aguilera M, Gruszecka-Kosowska A: Impact of cumulative environmental and dietary xenobiotics on human microbiota: risk assessment for one health. J Xenobiot 2022, 12(1): 56-63.
- Burgos-Aceves MA, Cohen A, Paolella G, Lepretti M, Smith Y, Faggio C, Lionetti L: Modulation of mitochondrial functions by xenobiotic-induced microRNA: from environmental sentinel organisms to mammals. Sci Total Environ 2018, 645: 79-88.
- Rock KD, Patisaul HB: Environmental mechanisms of neurodevelopmental toxicity. Curr Environ Health Rep 2018, 5(1): 145-157.
- Ehrlich P: Address in pathology, ON CHEMIOTHERAPY: delivered before the Seventeenth International Congress of Medicine. Br Med J 1913, 2(2746): 353-359.
- Shamul JG, Wang Z, Gong H, Ou W, White AM, Moniz-Garcia DP, Gu S, Clyne AM, Quiñones-Hinojosa A, He X: Meta-analysis of the make-up and properties of in vitro models of the healthy and diseased blood–brain barrier. Nat Biomed Eng 2024, Epub ahead of print.
- Kadry H, Noorani B, Cucullo L: A blood–brain barrier overview on structure, function, impairment, and biomarkers of integrity. Fluids Barriers CNS 2020, 17(1): 69.
- He Q, Liu J, Liang J, Liu X, Li W, Liu Z, Ding Z, Tuo D: Towards improvements for penetrating the blood–brain barrier—recent progress from a material and pharmaceutical perspective. Cells 2018, 7(4): 24.
- Zhang W, Liu QY, Haqqani AS, Leclerc S, Liu Z, Fauteux F, Baumann E, Delaney CE, Ly D, Star AT, et al: Differential expression of receptors mediating receptor-mediated transcytosis (RMT) in brain microvessels, brain parenchyma and peripheral tissues of the mouse and the human. Fluids Barriers CNS 2020, 17(1): 47.
- Greene C, Campbell M: Tight junction modulation of the blood brain barrier: CNS delivery of small molecules. Tissue Barriers 2016, 4(1): e1138017.
- Bentivoglio M, Kristensson K: Tryps and trips: cell trafficking across the 100-year-old blood–brain barrier. Trends Neurosci 2014, 37(6): 325-333.
- Bors LA, Erdő F: Overcoming the blood–brain barrier. challenges and tricks for CNS drug delivery. Scientia Pharmaceutica 2019, 87(1): 6.
- Wolburg H, Noell S, Mack A, Wolburg-Buchholz K, Fallier-Becker P: Brain endothelial cells and the glio-vascular complex. Cell Tissue Res 2009, 335(1): 75-96.
- Wu D, Chen Q, Chen X, Han F, Chen Z, Wang Y: The blood–brain barrier: structure, regulation, and drug delivery. Signal Transduct Target Ther 2023, 8(1): 217.
- Nagasawa K, Chiba H, Fujita H, Kojima T, Saito T, Endo T, Sawada N: Possible involvement of gap junctions in the barrier function of tight junctions of brain and lung endothelial cells. J Cell Physiol 2006, 208(1): 123-132.
- Roehlen N, Roca Suarez AA, El Saghire H, Saviano A, Schuster C, Lupberger J, Baumert TF: Tight junction proteins and the biology of hepatobiliary disease. Int J Mol Sci 2020, 21(3): 825.
- Archie SR, Al Shoyaib A, Cucullo L: Blood-brain barrier dysfunction in CNS disorders and putative therapeutic targets: an overview. Pharmaceutics 2021, 13(11): 1779.
- Yao S, Xu MD, Wang Y, Zhao ST, Wang J, Chen GF, Chen WB, Liu J, Huang GB, Sun WJ , et al: Astrocytic lactate dehydrogenase A regulates neuronal excitability and depressive-like behaviors through lactate homeostasis in mice. Nat Commun 2023, 14(1): 729.
- Mayo F, González-Vinceiro L, Hiraldo-González L, Calle-Castillejo C, Morales-Alvarez S, Ramírez-Lorca R, Echevarría M: Aquaporin-4 expression switches from white to gray matter regions during postnatal development of the central nervous system. Int J Mol Sci 2023, 24(3): 3048.
- Lien CF, Mohanta SK, Frontczak-Baniewicz M, Swinny JD, Zablocka B, Górecki DC: Absence of glial α-dystrobrevin causes abnormalities of the blood-brain barrier and progressive brain edema. J Biol Chem 2012, 287(49): 41374-41385.
- Engelhardt B, Liebner S: Novel insights into the development and maintenance of the blood–brain barrier. Cell Tissue Res 2014, 355(3): 687-699.
- Ezan P, André P, Cisternino S, Saubaméa B, Boulay A-C, Doutremer S, Thomas M-A, Quenech'Du N, Giaume C, Cohen-Salmon M: Deletion of astroglial connexins weakens the blood–brain barrier. J Cereb Blood Flow Metab 2012, 32(8): 1457-1467.
- Nagelhus EA, Ottersen OP: Physiological roles of aquaporin-4 in brain. Physiol Rev 2013, 93(4): 1543-1562.
- Kim DY, Zhang H, Park S, Kim Y, Bae C-R, Kim Y-M, Kwon Y-G: CU06-1004 (endothelial dysfunction blocker) ameliorates astrocyte end-feet swelling by stabilizing endothelial cell junctions in cerebral ischemia/reperfusion injury. J Mol Med (Berl) 2020, 98(6): 875-886.
- Zhao J, Sun J, Zheng Y, Zheng Y, Shao Y, Li Y, Fei F, Xu C, Liu X, Wang S , et al: Activated astrocytes attenuate neocortical seizures in rodent models through driving Na(+)-K(+)-ATPase. Nat Commun 2022, 13(1): 7136.
- Wolburg H, Wolburg-Buchholz K, Fallier-Becker P, Noell S, Mack AF: Structure and functions of aquaporin-4-based orthogonal arrays of particles. Int Rev Cell Mol Biol 2011, 287: 1-41.
- Liebner S, Czupalla CJ, Wolburg H: Current concepts of blood-brain barrier development. Int J Dev Biol 2011, 55(4)-5: 467-476.
- Daneman R, Zhou L, Kebede AA, Barres BA: Pericytes are required for blood–brain barrier integrity during embryogenesis. Nature 2010, 468(7323): 562-566.
- Brown LS, Foster CG, Courtney J-M, King NE, Howells DW, Sutherland BA: Pericytes and neurovascular function in the healthy and diseased brain. Front Cell Neurosci 2019, 13: 282.
- McConnell HL, Kersch CN, Woltjer RL, Neuwelt EA: The translational significance of the neurovascular unit. J Biol Chem 2017, 292(3): 762-770.
- Liao K, Niu F, Hu G, Buch S: Morphine-mediated release of astrocyte-derived extracellular vesicle miR-23a induces loss of pericyte coverage at the blood-brain barrier: Implications for neuroinflammation. Front Cell Dev Biol 2022, 10: 984375.
- Goncalves A, Antonetti DA: Transgenic animal models to explore and modulate the blood brain and blood retinal barriers of the CNS. Fluids Barriers CNS 2022, 19(1): 86.
- Jeske R, Albo J, Marzano M, Bejoy J, Li Y: Engineering brain-specific pericytes from human pluripotent stem cells. Tissue Engineering Part B: Reviews 2020, 26(4): 367-382.
- Lee SJ, Kim S, Jo DH, Cho CS, Kim SR, Kang D, Chae J, Yoo DK, Ha S, Chung J , et al: Specific ablation of PDGFRβ-overexpressing pericytes with antibody-drug conjugate potently inhibits pathologic ocular neovascularization in mouse models. Commun Med (Lond) 2021, 1: 58.
- Sengillo JD, Winkler EA, Walker CT, Sullivan JS, Johnson M, Zlokovic BV: Deficiency in Mural Vascular Cells Coincides with Blood–Brain Barrier Disruption in A lzheimer's Disease. Brain Pathol 2013, 23(3): 303-310.
- Wolburg H, Lippoldt A: Tight junctions of the blood–brain barrier: development, composition and regulation. Vascul Pharmacol 2002, 38(6): 323-337.
- Liu WY, Wang ZB, Zhang LC, Wei X, Li L: Tight junction in blood‐brain barrier: an overview of structure, regulation, and regulator substances. CNS Neurosci Ther 2012, 18(8): 609-615.
- Luissint A-C, Artus C, Glacial F, Ganeshamoorthy K, Couraud P-O: Tight junctions at the blood brain barrier: physiological architecture and disease-associated dysregulation. Fluids Barriers CNS 2012, 9(1): 1-12.
- Kwak M, Southard KM, Kim WR, Lin A, Kim NH, Gopalappa R, Lee HJ, An M, Choi SH, Jung Y , et al: Adherens junctions organize size-selective proteolytic hotspots critical for Notch signalling. Nat Cell Biol 2022, 24(12): 1739-1753.
- Malinova TS, Huveneers S: Sensing of cytoskeletal forces by asymmetric adherens junctions. Trends Cell Biol 2018, 28(4): 328-341.
- Turowski P, Kenny B-A: The blood-brain barrier and methamphetamine: open sesame? Front Neurosci 2015, 9: 156.
- Arya P, Haq SA: Effects of xenobiotics and their biodegradation in marine life. In: Smart bioremediation technologies. Epub ahead of print., edn.: Elsevier; 2019: 63-81.
- Štefanac T, Grgas D, Landeka Dragičević T: Xenobiotics—division and methods of detection: a review. J Xenobiot 2021, 11(4): 130-141.
- de Oliveira M, Frihling BEF, Velasques J, Magalhães Filho FJC, Cavalheri PS, Migliolo L: Pharmaceuticals residues and xenobiotics contaminants: occurrence, analytical techniques and sustainable alternatives for wastewater treatment. Sci Total Environ 2020, 705: 135568.
- Kelly GC, Watase CK, Ho DH: Blood-brain barrier function as a biomarker in toxicology: Impact of environmental toxicants. In: Biomarkers in Toxicology. Epub ahead of print., edn.: Springer; 2023: 583-607.
- Mortamais M, Pujol J, van Drooge BL, Macià D, Martínez-Vilavella G, Reynes C, Sabatier R, Rivas I, Grimalt J, Forns J , et al: Effect of exposure to polycyclic aromatic hydrocarbons on basal ganglia and attention-deficit hyperactivity disorder symptoms in primary school children. Environ Int 2017, 105: 12-19.
- Heidari Nejad S, Takechi R, Mullins BJ, Giles C, Larcombe AN, Bertolatti D, Rumchev K, Dhaliwal S, Mamo J: The effect of diesel exhaust exposure on blood–brain barrier integrity and function in a murine model. J Appl Toxicol 2015, 35(1): 41-47.
- Dauchy S, Miller F, Couraud P-O, Weaver RJ, Weksler B, Romero I-A, Scherrmann J-M, De Waziers I, Declèves X: Expression and transcriptional regulation of ABC transporters and cytochromes P450 in hCMEC/D3 human cerebral microvascular endothelial cells. Biochem Pharmacol 2009, 77(5): 897-909.
- Collins LL, Williamson MA, Thompson BD, Dever DP, Gasiewicz TA, Opanashuk LA: 2, 3, 7, 8-Tetracholorodibenzo-p-dioxin exposure disrupts granule neuron precursor maturation in the developing mouse cerebellum. Toxicol Sci 2008, 103(1): 125-136.
- Seelbach M, Chen L, Powell A, Choi YJ, Zhang B, Hennig B, Toborek M: Polychlorinated biphenyls disrupt blood–brain barrier integrity and promote brain metastasis formation. Environ Health Perspect 2010, 118(4): 479-484.
- Wang X, Hawkins BT, Miller DS: Aryl hydrocarbon receptor-mediated up-regulation of ATP-driven xenobiotic efflux transporters at the blood-brain barrier. FASEB J2011, 25(2): 644-652.
- Guo J: Transcription: the epicenter of gene expression. In., vol. 15: Springer; 2014: 409-411.
- Alberts B, Johnson A, Lewis J, Raff M, Roberts K, Walter P: An overview of gene control. Molecular Biology of the Cell 4th edition 2002, Epub ahead of print.
- Ralston A, Shaw K: Gene expression regulates cell differentiation. Nat Educ 2008, 1(1): 127-131.
- Gibney E, Nolan C: Epigenetics and gene expression. Heredity 2010, 105(1): 4-13.
- Hamilton JP: Epigenetics: principles and practice. Digestive diseases 2011, 29(2): 130-135.
- Mallick R, Duttaroy AK: Epigenetic modification impacting brain functions: Effects of physical activity, micronutrients, caffeine, toxins, and addictive substances. Neurochem Int 2023, Epub ahead of print.: 105627.
- Gabbianelli R: Modulation of the epigenome by nutrition and xenobiotics during early life and across the life span: the key role of lifestyle. Lifestyle Genom 2018, 11(1): 9-12.
- Bird A: Perceptions of epigenetics. Nature 2007, 447(7143): 396-398.
- Verma A, Maini J, Jain S, Ghasemi M, Kohli S, Brahmachari V: Epigenetic regulation and transcriptional memory in development; selection facilitating prudence. Int J Dev Biol 2020, 64(1-2-3): 181-201.
- Yin R, Mo J, Dai J, Wang H: Nickel (II) inhibits tet-mediated 5-methylcytosine oxidation by high affinity displacement of the cofactor iron (II). ACS Chem Biol 2017, 12(6): 1494-1498.
- Cheng TF, Choudhuri S, Muldoon‐Jacobs K: Epigenetic targets of some toxicologically relevant metals: a review of the literature. J Appl Toxicol 2012, 32(9): 643-653.
- Jiménez-Chillarón JC, Nijland MJ, Ascensão AA, Sardao VA, Magalhães J, Hitchler MJ, Domann FE, Oliveira PJ: Back to the future: transgenerational transmission of xenobiotic-induced epigenetic remodeling. Epigenetics 2015, 10(4): 259-273.
- Bar‐Or A, Nuttall RK, Duddy M, Alter A, Kim HJ, Ifergan I, Pennington CJ, Bourgoin P, Edwards DR, Yong VW: Analyses of all matrix metalloproteinase members in leukocytes emphasize monocytes as major inflammatory mediators in multiple sclerosis. Brain 2003, 126(12): 2738-2749.
- Wu S, Liu H, Zhao H, Wang X, Chen J, Xia D, Xiao C, Cheng J, Zhao Z, He Y: Environmental lead exposure aggravates the progression of Alzheimer's disease in mice by targeting on blood brain barrier. Toxicol Lett 2020, 319: 138-147.
- Ding J, Sun B, Gao Y, Zheng J, Liu C, Huang J, Jia N, Pei X, Jiang X, Hu S, et al: Evidence for chromium crosses blood brain barrier from the hypothalamus in chromium mice model. Ecotoxicol Environ Saf 2024, 273: 116179.
- Takahashi T, Fujimura M, Koyama M, Kanazawa M, Usuki F, Nishizawa M, Shimohata T: Methylmercury causes blood-brain barrier damage in rats via upregulation of vascular endothelial growth factor expression. PLoS One 2017, 12(1): e0170623.
- Ho DH, Burggren WW: Blood-brain barrier function, cell viability, and gene expression of tight junction-associated proteins in the mouse are disrupted by crude oil, benzo [a] pyrene, and the dispersant COREXIT. Comparati Biochemi and Physiol Part C: Toxicol & Pharmacol 2019, 223: 96-105.
- Hao W, Hao C, Wu C, Xu Y, Wu S, Lu X, Yang J, Jin C: Aluminum impairs cognitive function by activating DDX3X-NLRP3-mediated pyroptosis signaling pathway. Food Chem Toxicol 2021, 157: 112591.
- Collins NT, Cummins PM, Colgan OC, Ferguson G, Birney YA, Murphy RP, Meade G, Cahill PA: Cyclic Strain–Mediated Regulation of Vascular Endothelial Occludin and ZO-1: Influence on Intercellular Tight Junction Assembly and Function. Arterioscler Thromb Vasc Biol 2006, 26(1): 62-68.
- Tobwala S, Wang H-J, Carey JW, Banks WA, Ercal N: Effects of lead and cadmium on brain endothelial cell survival, monolayer permeability, and crucial oxidative stress markers in an in vitro model of the blood-brain barrier. Toxics 2014, 2(2): 258-275.
- Liu X, Su P, Meng S, Aschner M, Cao Y, Luo W, Zheng G, Liu M: Role of matrix metalloproteinase-2/9 (MMP2/9) in lead-induced changes in an in vitro blood-brain barrier model. Int J Biol Sci 2017, 13(11): 1351-1360.
- Yamauchi H, Hitomi T, Takata A: Evaluation of arsenic metabolism and tight junction injury after exposure to arsenite and monomethylarsonous acid using a rat in vitro blood–Brain barrier model. Plos one 2023, 18(11): e0295154.
- Branca JJV, Maresca M, Morucci G, Mello T, Becatti M, Pazzagli L, Colzi I, Gonnelli C, Carrino D, Paternostro F, et al: Effects of Cadmium on ZO-1 Tight Junction Integrity of the Blood Brain Barrier. Int J Mol Sci 2019, 20(23): 6010.
- Kwon HS, Koh S-H: Neuroinflammation in neurodegenerative disorders: the roles of microglia and astrocytes. Transl Neurodegener 2020, 9(1): 42.
- Huang J, Li C, Shang H: Astrocytes in neurodegeneration: inspiration from genetics. Front Neurosci 2022, 16: 882316.
- Kushwaha R, Mishra J, Tripathi S, Khare P, Bandyopadhyay S: Arsenic, cadmium, and lead like troglitazone trigger PPARγ-dependent poly (ADP-ribose) polymerase expression and subsequent apoptosis in rat brain astrocytes. Mol Neurobiol 2018, 55(3): 2125-2149.
- Wang Q, Luo W, Zhang W, Liu M, Song H, Chen J: Involvement of DMT1+ IRE in the transport of lead in an in vitro BBB model. Toxicol in Vitro 2011, 25(4): 991-998.
- Motawei SM, Sudha T, Yalcin M, Godugu K, Mousa SA: Lead-induced endothelial cell dysfunction: protective effect of sulfated non-anticoagulant low molecular weight heparin. Toxicol and Environ Health Sci 2021, 13: 123-131.
Asia-Pacific Journal of Pharmacotherapy & Toxicology
p-ISSN: 2788-6840
e-ISSN: 2788-6859
Copyright © Asia Pac J Pharmacother Toxicol. This work is licensed under a Creative Commons Attribution-NonCommercial-No Derivatives 4.0 International (CC BY-NC-ND 4.0) License.